News Article
Uniform thermal control
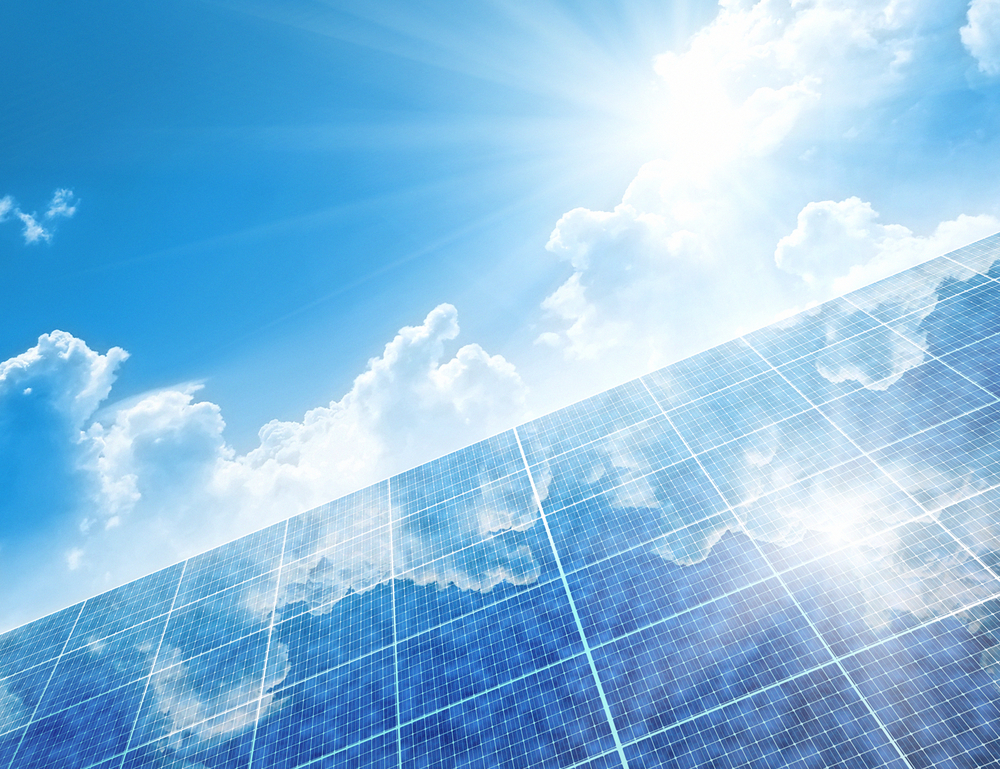
Continual improvement is the usual mantra for technology manufacturing and PV is no different. Dr. Hans Bell , Milo Gaggioli and Manuel Schwarzenbolz of Rehm Thermal Systems, along with Dr. Kay Reuter, Mathias Ribke, Dr. Norbert Müller, Christian Arndt and Dr. Armin Froitzheim of Bosch Solar Energy come together to reveal recent results for machine capability of firing systems.
Firing is a production process during which the contacts of a crystalline solar cell are conclusively formed. It's a thermal process that takes place in a tunnel oven, in which the solar wafers are exposed to a defined temperature profile. The tolerance width and the reproducibility of this thermal process are essential criteria for assuring uniform quality of the manufactured solar cells. The temperature profile (see figure 1) is subdivided into several zones.

Figure 1: Temperature profile of a firing oven.
The solar wafers, which are printed with a thick-layer paste, are heated up to a temperature of approximately 500 to 600° C in the burnout zone. The goal of this process step is to drive out the nonvolatile organic constituents which remain in the paste after the upstream drying process. The solar pastes are sintered in the firing zone (approx. 900° C), where the electrical contact is generated. The firing process is especially decisive for the quality of the solar cells. The purpose of the cooling zone is to cool the solar cells down to room temperature.
A complex relationship exists between the utilized solar pastes, the temperature gradients, the absolute temperatures, the wafer material and, in particular, the quality of the solar cell coating system. A great deal of experience and process knowledge is necessary in order to define the temperature profile that results in the greatest degree of efficiency for the manufactured solar cells. Measurement of temperature profiles presents a number of challenges in this respect. Amongst other factors, this has to do with the relatively high temperatures in the firing zone, as well as the very steep temperature gradients that occur from segment to segment within the temperature profile.
A further problem results from the relatively minimal thermal mass of a solar cell as compared with the measuring equipment. Even a slightly altered thermal mass at the measuring point (caused for example by unsuitable mounting of a thermocouple) can influence the measured temperature. Limit deviation, calibration and the ageing characteristics of the thermocouples, as well as test setup and execution, must also be taken into consideration.
On the one hand, this article discusses the measurement of temperature profiles, and it specifically addresses the question of which acquired temperature data are stable enough for the calculation of machine capability coefficients. In addition to theoretical observations, results are also presented which have been obtained during close collaboration between Bosch Solar Energy AG and Rehm Thermal Systems GmbH.
Machine capability
Process capability is defined by DIN ISO 21747:2006 [1] as a statistical estimate of the consequence of a characteristic of a process which is verifiably kept under control, wherein the estimated value describes the capability of the process to affect the characteristic such that it fulfills its respective requirement. Sauer [2] goes into the subject of the quality capability of processes in great detail. Process capability and machine capability describe the potential capability of a process (a machine) to produce a certain characteristic in a constant fashion within specified tolerance limits. Capable machines are a prerequisite for capable processes. Machine capability only takes the capability of the machine into consideration [2]. The capability of a process/machine can be numerically described through the use of generally accepted capability coefficients.
Due to the fact that process capability depends upon the capability of the utilized production machines, higher numeric requirements are specified for machine capability. Sauer [2] indicates that in general, a capability value of Cmk > 1.67 is required for machines.
Wohlrabe[4] describes the fundamental procedure used for capability analysis. Generally speaking, at least 50 individual measurements are required.
Problems become plainly apparent right from the start when attempting to ascertain capability coefficients for the firing process used for crystalline solar cells. Which measurable characteristics can be defined? The quality of the manufactured solar cells depends upon a great variety of influencing factors (e.g. wafer quality, POCl3 process, solar paste combination etc.), and the impact of the machine (firing system) accounts for only a small portion of overall influence. Consequently, cell parameters are unsuitable as a measurable characteristic of machine capability.
Temperature, conveyor speed and, if applicable, temperature differences are all possible candidates as measurable characteristics.
Conveyor speed is generally not used for the ascertainment of machine capability data. To some extent this is due to the knowledge that minimal variation in conveyor speed has nearly no significant effect on cell parameters. At the relatively high speeds typical of the firing process (5.5 to 6.0 meters per minute), the amount of heat absorbed by the individual wafers is nearly identical when the usual conveyor speed tolerances are taken into account (< 0.5%, ~ ± 3.5 cm/min.). A comparison of the temperature profiles for various conveyor speeds differing by ± 25 cm/min. (550 to 600 cm/min.) is shown in figure 2. The heat equivalent absorbed by the solar wafer in the peak zone deviates by no more than ± 4.5% of the mean value, although the difference between maximum temperatures is 14.5° C.

Figure 2: Influence of conveyor speed in the peak zone.
We take a critical view of acquiring temperature differences for the purpose of calculating machine capability coefficients. This is discussed briefly below. The temperature difference between two measuring points, as shown in figure 3 is noteworthy.

Figure 3: Calculation of temperature difference on the solar cell.
As a prerequisite, the wafer must be very well prepared with several thermocouples. Differences in thermocouple mounting result in changes to the thermal mass at the measuring point, which has a critical influence on data acquisition. The position of the test wafer on the conveyor belt influences thermal mass as well.
Furthermore, the results only reflect the temperature difference on a single wafer (e.g. ôL-Rô), which depends more upon wafer preparation than on interaction with the firing systems temperature profile. Table 1 lists the statistical values associated with figure 3. The requirement for a capability coefficient of Cm > 1.67 for a tolerance width of ± 7 K is fulfilled using temperature difference on the wafer as a characteristic, although this is not the case when absolute temperature on the same test wafer is used as the characteristic.

Temperature Difference ôL-Rô | Absolute Temperature (R) | |
Mean value | 5.5 | 771.8 |
Standard deviation | 2 | 1.7 |
Machine capability Cm | 1.72 | 1.18 |
Table 1: Statistical values associated with figure 3.
For this reason, we haven't made use of this temperature difference for the capability analysis. The acquisition of temperature differences between lanes is used for dual and multi-lane systems.

Figure 4: Calculation of standard deviation in dual lane systems.
Standard deviation of all measuring points on the test board for both the right and left-hand lanes is used as a basis for calculation in figure 4. Heat transfer tolerance over the entire width of the profile is ascertained in this way. Due to the fact that only one test board is available as a rule, the lanes have to be measured one after the other with the same board. This, too, is not in the spirit of the capability analysis. The calculation based on the procedure depicted in figure 5 provides us with the mean temperature difference between the lanes. In this case as well, two test boards which run through the oven simultaneously are really required.

Figure 5: Calculation of the mean value in dual lane systems.
In our opinion, measurement of absolute temperatures makes more sense for the ascertainment of machine capability coefficients. A firing system offers numerous options for acquiring temperatures. Figure 6 depict the temperature curves generated by temperature measuring systems integrated into the machine.

Figure 6: Peak (zone) temperature acquired by the firing system's internal thermocouples.
The thermocouples designated as controllers are incorporated into the firing system's temperature control loop, whereas the so-called watchdog thermocouples measure temperature in proximity to the conveyor belt independent of the control system. Table 2 lists the corresponding statistical values. Machine capability Cm is calculated here with a tolerance width of ± 5 K.

Controller | Watchdog | |
Mean value | 905.4 | 886.6 |
Standard deviation | 0.9 | 9.8 |
Machine capability Cm | 1.78 | 0.17 |
Table 2: Statistical values (peak zone Tmax) associated with figure 6.
If a controller thermocouple is used to determine machine capability coefficients, the required value of Cm > 1.67 is easily complied with, whereas a dramatically worse value results from the data supplied by the watchdog thermocouple. This comparison demonstrates that it makes little sense to use data from thermocouples which are integrated into the firing system in order to calculate machine capability coefficients.
Measuring equipment and tolerance width
For this reason, it's advisable to ascertain absolute temperatures on standardized test boards, which are independent of the firing system, when determining machine capability coefficients. These can be either calibrated test setups on specified solar wafers (as shown in figure 7a) or standardized measuring devices consisting of, for example, defined metal sheets to which thermocouples are attached (see figure 7b). The latter have the advantage of being significantly more rugged, and are thus able to withstand the procedure which involves multiple repeat measurements.

Figure 7a: Test board with solar wafer and two thermocouples.

Figure 7b: Test board made of specified metal sheet.
As opposed to mechanical systems, the fact that there's direct interaction between the firing system and the measuring device (test board) must be taken into consideration in the real thermodynamic world. Fundamentally, firing systems are radiation systems, i.e. heat transfer is subject to the conditions set forth in the Stefan-Boltzmann law. Emittance specifies the amount of radiated power emitted/absorbed by the respective body. Actual emittance depends to a great extent on the respective material (color and finishing quality of the surface), and usually lies within a range of 0.012 to 0.98.

Figure 8 depicts measurements conducted by Vogg [3] on various solar wafers. Settings used at the radiation system were identical for all of the measurements. The significantly varying temperature-time curves make is plainly apparent that not just any crystalline solar wafers with various surfaces are suitable as measuring devices.

Figure 8: Comparison of heat absorption at various solar wafers.
The next difficulty arises in establishing tolerance limits To and Tu. All too frequently, experience from the field of mechanical engineering is used to this end, and excessively tight tolerance limits are specified which cannot be adhered to in light of the thermodynamic circumstances which prevail in an inline system operated at roughly 1000° C. Consequently, table 3 below should be used as a basis for discussing which elements will determine overall tolerance width To - Tu.
Test Board | [K] |
Thermocouple on the test board | ± 4.0 |
Data logger | ± 0.5 |
Firing System | |
Thermocouples in the control loop | ± 4.0 |
Controller per heat zone | ± 0.5 |
Cross profile (dTw) | ± 2.0 |
Tolerance Width | ± 11.0 |
Table 3: Tolerances.
Temperatures are determined by means of a test board to which type K thermocouples with a limit deviation of ± 4.0 K (± 0.004à—T) are attached in accordance with DIN EN 60584-2. Data are recorded to a data logger during the measurement procedure with a measuring accuracy of ± 0.5 K. The test board and the firing system interact with each other, which means that the repetition accuracy of the firing system must also be taken into consideration.
The controllers for the individual heat zones have an accuracy of ± 0.5 K. Finally, in the case of multi-lane systems, heat transfer homogeneity must also be taken into account, which is represented by the numeric value of cross profile dTw (also known as the width profile). In actual manufacturing practice it has to be irrelevant in which lane the solar cells are produced, and uniform quality should be expected in any case. Cross profile dTw is typically ± 2.5 K.
Figure 9 shows the characteristic time curves for temperatures in the peak zone for two lanes of a firing system, which have been ascertained with Rehm's Cross-Profiler measuring system. The Cross-Profiler makes it possible to perform measurements over a lengthy period of time at the same place within the firing system's peak zone.

Figure 9: Cross profile of a dual lane firing system.
Under certain circumstances it may be important to always record a full revolution of the conveyor belt, because the belt itself has significant influence on the measured temperature curve. Table 4 lists the corresponding data. Maximum standard deviation (rounded off) has been taken into consideration for the cross profile.
All in all, this often results in a tolerance width which is quite large in comparison with mechanical systems. In actual practice, however, this type of procedure has turned out to be a solid basis for ascertaining capability values for the firing process.
Field results
Machine capability analyses were conducted on an RFS-D-250 single-lane firing oven manufactured by Rehm Thermal Systems GmbH with unchanging oven settings (temperature, conveyor speed, exhaust air, supply air). A calibrated test board was used to this end consisting of a defined metal sheet (see figure 7b) with 2 type K thermocouples. A Datapaq Q18 data logger was used to record absolute temperature data. 50 oven temperature profiles were recorded for each of the following three loading statuses with this test setup. Maximum absolute temperature Tmax in the firing zone was determined with the help of Datapaq InsightSolar Tracker software.
1 Empty oven
2 Partially loaded oven
3 Fully loaded oven
These three load variants are intended to reflect various possible production statuses in the field of solar cell manufacturing. Empty means that the oven was not loaded with cells for a period of 10 minutes, after which it was profiled. Partial loading of the oven occurs during the production process when continuous loading of the oven cannot be assured due to the upstream silk-screening process (e.g. due to removal of broken cells).
This status was simulated by not loading the oven for a period of 8 minutes and then releasing 50 wafers into the oven. 30 seconds after the last wafer entered the oven, the oven was profiled with the help of the test setup. In order to record the temperature profile in the fully loaded state, the oven was loaded with 50 cells and the test setup was transported into the oven immediately thereafter. 50 more cells were fed to the oven directly after the test board.
Evaluation of the resulting data reveals that maximum temperature in the peak zone can be assumed to be nearly normally distributed for each loading status. Figure 10 shows the probability grid of the left-hand thermocouple in the fully loaded state as an example.
Under these conditions, it's possible to calculate as of which tolerance width a machine capability of Cmk > 1.67 can be achieved for the various loading statuses. The overview in table 5 shows the calculated tolerance widths of the thermocouples for the various loading statuses.

Figure 10: Probability grid for temperature measured at the left thermocouple.
It was possible to substantiate that the firing system fulfills machine capability requirements for an overall tolerance width of ± 7.5 to ± 9.5 K. The discussion in section 3 demonstrates that deviations of ± 9 K can be expected for the utilized single-lane fast firing oven due to the utilized measuring device and its interaction with the firing system. This indicates that the tolerance width determined by means of the machine capability analysis lies within the tolerances of the test setup.
From a process engineering standpoint, it makes good sense to conduct machine capability analyses on the basis of parameters whose tolerances lead us to expect large fluctuations of the solar cells' characteristic electrical values. From our point of view, maximum temperature in the firing zone is best suited for this purpose. The characteristic electrical values are decisively determined by the attributes of the semiconductor-metal contact.
Contact formation is influenced by complex cause-and-effect relationships between the cell material (paste selection, passivation layers etc.) and the firing system (firing profile, conveyor speed etc.) [6, 7]. The firing profile is characterized in turn by maximum temperature in the firing zone [8].
References
[1] DIN ISO 21747:2006, Statistical methods "“ Process performance and capability statistics for measured quality characteristics, DIN Deutsches Institut für Normung e.V (German Institute for Standardization), p. 24
[2] W. Sauer, Prozesstechnologie der Elektronik, Hanser Verlag 2003, pp. 282 ff
[3] Florian Vogg, work-study thesis prepared at Rehm Thermal Systems, Blaubeuren, Germany, 2010
[4] Heinz Wohlrabe, Maschinen- und Prozessfähigkeit von Bestückausrüstungen der SMT, pp. 35 ff, Verlag Markus Detert, 2000, ISBN 3-934142-01-X
[5] Thermocouples - Part 2: Tolerances (IEC 60584-2:1982 + A1:1989); German version EN 60584-2:1993
[6] Schubert G., Thick Film Metallisation of Crystalline Silicon Solar Cells, dissertation, University at Konstanz, Germany, 2006
[7] Kwon T. et al, The effect of firing temperature profiles for the high efficiency of crystalline Si solar cells, Solar Energy Materials & Solar Cells, 823-829, 2010
[8] Schütt et al, Local characterization of co-firing-induced inhomogeneities of conventional MC-Si solar cells, Proceedings of 28th European Photovoltaic Solar Energy Conference and Exhibition, Paris, 2013